Peering into a Quantum Well
Despite being cool, ultra-efficient, and long-lasting, solid-state lighting has yet to conquer the general lighting market partly due to a problem called "efficiency droop." New findings from simulations carried out at Lawrence Berkeley National Laboratory's National Energy Research Scientific Computer Center (NERSC) have unearthed droop's elusive cause, illuminating a key barrier to this potentially energy-saving alternative to conventional incandescent and fluorescent lighting.
At issue, in particular, are the blue (and green) nitride-based light-emitting diodes (LEDs) that are used—in combination with yellow phosphor—to produce solid-state white light. They hold the key to replacing conventional bulbs. These LEDs are already widely used to backlight laptop screens and smart phone displays, in indicator lighting on dashboards, and for countless other low-power applications throughout the economy. At low power, these nitride-based LEDs are exceptionally efficient; but pump up the current to room-illuminating levels and efficiency takes a nosedive.
"If you use more electricity, you do get more light, but the efficiency isn't as good, and that adds to the higher cost of LED-based lighting," says Emmanouil Kioupakis, a researcher at the University of California Santa Barbara (UCSB) and lead author of the study. To probe the mystery of efficiency droop, the researchers relied on detailed, quantum-mechanical simulations of the inner-workings of nitride-based LEDs.
The new study doesn't solve the problem of droop, but it does set researchers and engineers on the right path to do so, says Chris Van de Walle, head of the UCSB research group that carried out the work: "Identifying the root cause of the problem is an indispensable first step toward devising solutions."
The Promise of Solid-State Lighting
Today, lighting accounts for around 20 percent of the average U.S. household's electrical consumption. That's not very surprising when you consider that over 80 percent of the light bulbs sold today are incandescent, a technology that has changed little since its 1880 commercial debut.
Incandescent lights work by heating a wire until it glows, converting far more power into heat than light. As a result, the garden-variety light bulb only produces about 16 lumens per watt (lm/W). A lumen is roughly equal to the amount of light the human eye perceives from one candle about one foot away as it falls on a square foot of area.
Theoretically, LED-based lights can produce up to 300 lm/W. That's about three-times better than today's most energy-efficient alternative, compact fluorescent lights (CFLs). Because of droop, however, today's high-power LED lights perform about the same or only slightly better than CFLs.
A Department of Energy study projects that improved efficiency and increased use of LED lights over the two decades between 2010 and 2030 could save 1,488 terawatt-hours of electricity. That's a savings of $120 billion. It would also spare the atmosphere an estimated 246 million metric tons of carbon dioxide emissions over the same period.
Adding to the urgency of overcoming LED droop is the fact that a growing number of nations have banned the sale of wasteful incandescent lights in coming years. Although LEDs last longer, operate more coolly, and don't pose the disposal hazards of compact fluorescent lights—which contain the heavy metal mercury—they also cost up to 10 times as much as CFLs. To offer a viable alternative, high-power LED lighting has to save enough energy to convince consumers it is worth a bigger up-front investment.
Mystery of the Missing Photons
At the heart of every LED is a multi-layered microchip. In the case of blue and green LEDs, the base semiconductor material is gallium nitride, so the entire family is called nitride-based LEDs.
One outer layer of this semiconductor sandwich is doped with an element that has just one more electron on its outer shell than the atom it is replacing in the semiconductor's crystal lattice (gallium). Because of the overabundance of negatively charged electrons, this is called an n-type semiconductor.
The other slice of bread in this metaphorical sandwich is a p-type (positive-type) semiconductor. It is doped with an element that has one less electron on its outer shell than the gallium atom it replaces in the lattice. The missing electrons create what physicists call an electron hole, usually just termed a hole, with a positive charge.
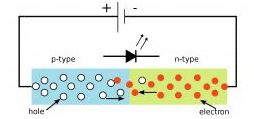
Image courtesy of Lawrence Berkeley National Laboratory
Schematic of a light-emitting diode. Light is emitted when an electron “recombines” with a hole.
When current is applied across the chip, the "extra" electrons and holes are pushed into a sandwich of very thin layers between these two outer wafers. Here they are trapped in a space so small that the weird rules of quantum physics reign: a quantum well.
When an electron and hole combine in this well, out pops a photon or wave-particle of light. Apply more electricity and you get more photons. At least, that's what happens in red, yellow, and orange LEDs.
The exact cause of drooping photon production in nitride-based LEDs has been hotly debated between two main camps: One thinks the electrons become so energized that they overflow the quantum well and escape. The other camp, to which Van de Walle's group belongs, thinks that the energy created when an electron and hole combine—physicists call this interaction a "recombination"—is being converted into an electron or a hole rather than a photon. When the LED is operating properly, the recombination yields a photon. But as the current increases, the recombinations increasingly yield an electron or hole instead of a photon. The latter phenomenon is called "Auger recombination," named for the French physicist Pierre Victor Auger, who discovered it back in 1923.
Cracking the Case
In this study, Kioupakis and colleagues simulated the semiconductor alloys involved in LEDs, a computationally intense process. Using up to 4,000 processor cores at once and a total of a million processor core hours, the team calculated from first principles the interactions of charge carriers involved under different conditions for different loss mechanisms.
"Because of the vast number of possible beginning and ending states, these calculations are very challenging," says Kioupakis. "That's why we used NERSC; its highly parallel Franklin and Hopper [supercomputer] systems offer the kind of power we needed. We couldn't have done it otherwise," he explains.
Their results not only confirmed the importance of Auger recombination, but they also uncovered an unsuspected accomplice: carrier scattering. Essentially, because of imperfections in the crystal lattice of the gallium nitride and various interactions with heat, the electrons begin bouncing around irregularly and colliding with holes. Instead of producing photons, these disorganized collisions or recombinations produce electrons or holes, and the energy eventually dissipates as heat.
"This carrier scattering opens up additional recombination channels where the energy is eventually lost to heat" Kioupakis explains. In fact, the effects of this "indirect" Auger recombination were found to be about 1,000 times stronger than the direct process, the team found.

Image courtesy of Lawrence Berkeley National Laboratory
This illustration shows what happens in nitride-based LEDs. At left, an electron (negative) and electron hole (positive) recombine and release light (left). In Auger recombination (right) the electron and hole combine with a third carrier, releasing no photon in the process. In this case, the energy loss is also assisted by indirect processes, vibrations in the crystal lattice shown as squiggles.
Illuminating the Path Ahead
The team's research suggests ways engineers might work around the problem, but there's no way to solve it directly since the conditions producing Auger recombination are intrinsic to nitride-based LEDs. "As long as you have a lot of carriers interacting with each other, you are going to have Auger recombination," Kioupakis says.
Some of the methods suggested by the research—lowering carrier density by widening the quantum well or growing the devices along different crystallographic directions, for example—are already being explored by researchers and industry.
"With Auger recombination now established as the culprit, we can focus on creative approaches to suppress or circumvent this loss mechanism," Van de Walle says.
The research was funded in part by the Center for Energy Efficient Materials, a DOE Energy Frontier Research Center, which is led by the University of California, Santa Barbara, and supported by the DOE Office of Science, which also supports NERSC. The California NanoSystems Institute's Computing Facility at UCSB and the National Science Foundation-funded TeraGrid also provided computing resources for this study.
—Margie Wylie, Lawrence Berkeley National Laboratory, mwylie@lbl.gov
Funding
Research: DOE Office of Science, Office of Basic Energy Sciences
Facility (NERSC): DOE Office of Science, Office of Advanced Scientific Computing Research
Publication
E. Kioupakis et al., "Indirect Auger recombination as a cause of efficiency droop in nitride light-emitting diodes," Applied Physics Letters 98, 161107 (2011)